7.0: Introduction
Changes in precipitation are one of the most important potential outcomes of a warming world because precipitation is integral to the very nature of society and ecosystems. These systems have developed and adapted to the past envelope of precipitation variations. Any large changes beyond the historical envelope may have profound societal and ecological impacts.
Historical variations in precipitation, as observed from both instrumental and proxy records, establish the context around which future projected changes can be interpreted, because it is within that context that systems have evolved. Long-term station observations from core climate networks serve as a primary source to establish observed changes in both means and extremes. Proxy records, which are used to reconstruct past climate conditions, are varied and include sources such as tree ring and ice core data. Projected changes are examined using the Coupled Model Intercomparison Project Phase 5 (CMIP5) suite of model simulations. They establish the likelihood of distinct regional and seasonal patterns of change.
7.1: Historical Changes
7.1.1 Mean Changes
Annual precipitation averaged across the United States has increased approximately 4% over the 1901–2015 period, slightly less than the 5% increase reported in the Third National Climate Assessment (NCA3) over the 1901–2012 period.1 There continue to be important regional and seasonal differences in precipitation changes (Figure 7.1). Seasonally, national increases are largest in the fall, while little change is observed for winter. Regional differences are apparent, as the Northeast, Midwest, and Great Plains have had increases while parts of the Southwest and Southeast have had decreases. The slight decrease in the change in annual precipitation across the United States since NCA3 appears to be the result of the recent lingering droughts in the western and southwestern United States.2 ,3 However, the recent meteorological drought in California that began in late 20114 ,5 now appears to be largely over, due to the substantial precipitation and snowpack the state received in the winter of 2016–2017. The year 2015 was the third wettest on record, just behind 1973 and 1983 (all of which were years marked by El Niño events). Interannual variability is substantial, as evidenced by large multiyear meteorological and agricultural droughts in the 1930s and 1950s.
Figure 7.1
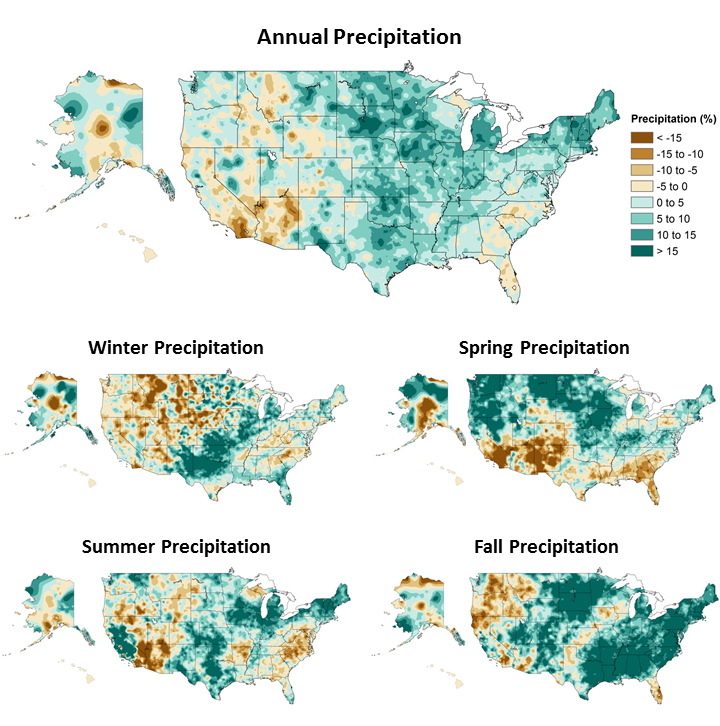
Annual and seasonal changes in precipitation over the United States. Changes are the average for present-day (1986–2015) minus the average for the first half of the last century (1901–1960 for the contiguous United States, 1925–1960 for Alaska and Hawai‘i) divided by the average for the first half of the century. (Figure source: [top panel] adapted from Peterson et al. 2013,78 © American Meteorological Society. Used with permission; [bottom four panels] NOAA NCEI, data source: nCLIMDiv].
Changes in precipitation differ markedly across the seasons, as do regional patterns of increases and decreases. For the contiguous United States, fall exhibits the largest (10%) and most widespread increase, exceeding 15% in much of the Northern Great Plains, Southeast, and Northeast. Winter average for the United States has the smallest increase (2%), with drying over most of the western United States as well as parts of the Southeast. In particular, a reduction in streamflow in the northwestern United States has been linked to a decrease in orographic enhancement of precipitation since 1950.6 Spring and summer have comparable increases (about 3.5%) but substantially different patterns. In spring, the northern half of the contiguous United States has become wetter, and the southern half has become drier. In summer, there is a mixture of increases and decreases across the Nation. Alaska shows little change in annual precipitation (+1.5%); however, in all seasons, central Alaska shows declines and the panhandle shows increases. Hawai‘i shows a decline of more than 15% in annual precipitation.
7.1.2 Snow
Changes in snow cover extent (SCE) in the Northern Hemisphere exhibit a strong seasonal dependence.7 There has been little change in winter SCE since the 1960s (when the first satellite records became available), while fall SCE has increased. However, the decline in spring SCE is larger than the increase in fall and is due in part to higher temperatures that shorten the time snow spends on the ground in the spring. This tendency is highlighted by the recent occurrences of both unusually high and unusually low monthly (October–June) SCE values, including the top 5 highest and top 5 lowest values in the 48 years of data. From 2010 onward, 7 of the 45 highest monthly SCE values occurred, all in the fall or winter (mostly in November and December), while 9 of the 10 lowest May and June values occurred. This reflects the trend toward earlier spring snowmelt, particularly at high latitudes.8 An analysis of seasonal maximum snow depth for 1961–2015 over North America indicates a statistically significant downward trend of 0.11 standardized anomalies per decade and a trend toward the seasonal maximum snow depth occurring earlier—approximately one week earlier on average since the 1960s.8 There has been a statistically significant decrease over the period of 1930–2007 in the frequency of years with a large number of snowfall days (years exceeding the 90th percentile) in the southern United States and the U.S. Pacific Northwest and an increase in the northern United States.9 In the snow belts of the Great Lakes, lake effect snowfall has increased overall since the early 20th century for Lakes Superior, Michigan-Huron, and Erie.10 However, individual studies for Lakes Michigan11 and Ontario12 indicate that this increase has not been continuous. In both cases, upward trends were observed until the 1970s/early 1980s. Since then, however, lake effect snowfall has decreased in these regions. Lake effect snows along the Great Lakes are affected greatly by ice cover extent and lake water temperatures. As ice cover diminishes in winter, the expectation is for more lake effect snow until temperatures increase enough such that much of what now falls as snow instead falls as rain.13 ,14
End-of-season snow water equivalent (SWE)—especially important where water supply is dominated by spring snow melt (for example, in much of the American West)—has declined since 1980 in the western United States, based on analysis of in situ observations, and is associated with springtime warming.15 Satellite measurements of SWE based on brightness temperature also show a decrease over this period.16 The variability of western United States SWE is largely driven by the most extreme events, with the top decile of events explaining 69% of the variability.17 The recent drought in the western United States was highlighted by the extremely dry 2014–2015 winter that followed three previous dry winters. At Donner Summit, CA, (approximate elevation of 2,100 meters) in the Sierra Nevada Mountains, end-of-season SWE on April 1, 2015, was the lowest on record, based on survey measurements back to 1910, at only 0.51 inches (1.3 cm), or less than 2% of the long-term average. This followed the previous record low in 2014. The estimated return period of this drought is at least 500 years based on paleoclimatic reconstructions.18
7.1.3 Observed changes in U.S. seasonal extreme precipitation.
Extreme precipitation events occur when the air is nearly completely saturated. Hence, extreme precipitation events are generally observed to increase in intensity by about 6% to 7% for each degree Celsius of temperature increase, as dictated by the Clausius–Clapeyron relation. Figure 7.2 shows the observed change in the 20-year return value of the seasonal maximum 1-day precipitation totals over the period 1948–2015. A mix of increases and decreases is shown, with the Northwest showing very small changes in all seasons, the southern Great Plains showing a large increase in winter, and the Southeast showing a large increase in the fall.
Figure 7.2
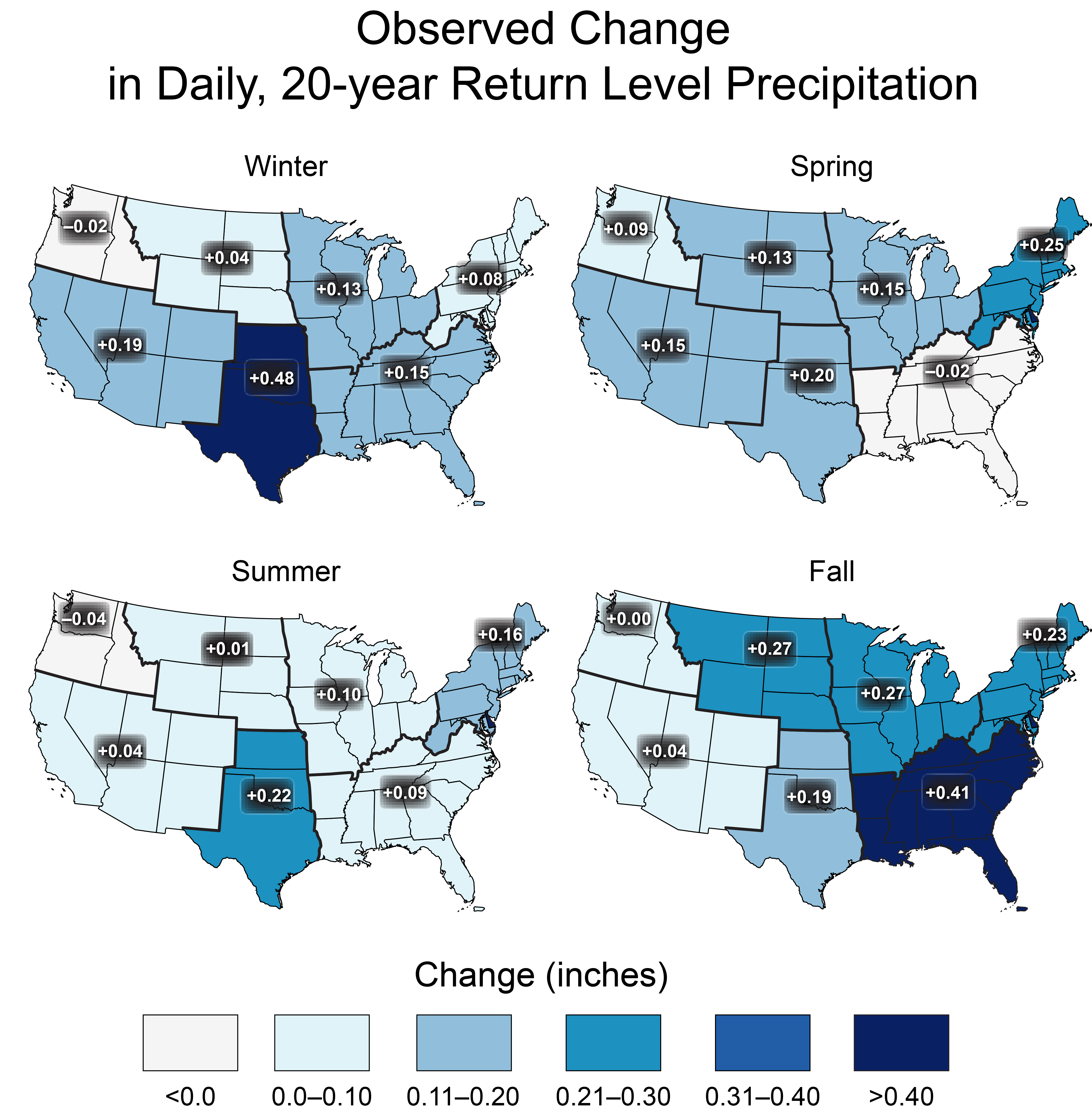
Observed changes in the 20-year return value of the seasonal daily precipitation totals for the contiguous United States over the period 1948 to 2015 using data from the Global Historical Climatology Network (GHCN) dataset. (Figure source: adapted from Kunkel et al. 2013;61 © American Meteorological Society. Used with permission.)
A U.S. index of extreme precipitation from NCA3 was updated (Figure 7.3) through 2016. This is the number of 2-day precipitation events exceeding the threshold for a 5-year recurrence. The values were calculated by first arithmetically averaging the station data for all stations within each 1° by 1° latitude/longitude grid for each year and then averaging over the grid values across the contiguous United States for each year during the period of 1896–2015. The number of events has been well above average for the last three decades. The slight drop from 2006–2010 to 2011–2016 reflects a below-average number during the widespread severe meteorological drought year of 2012, while the other years in this pentad were well above average. The index value for 2015 was 80% above the 1901–1960 reference period average and the third highest value in the 120 years of record (after 1998 and 2008).
Figure 7.3
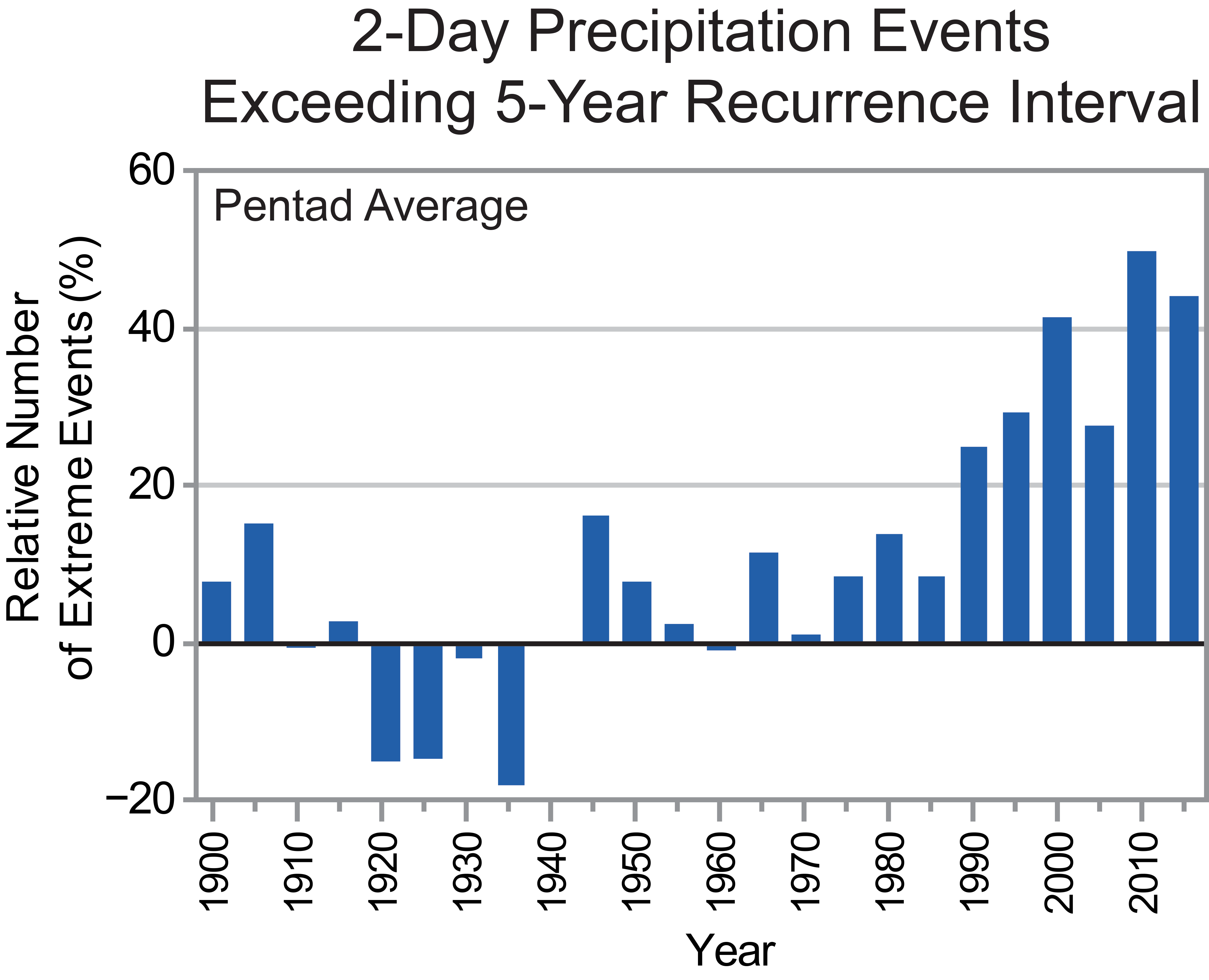
Index of the number of 2-day precipitation events exceeding the station-specific threshold for a 5-year recurrence interval in the contiguous United States, expressed as a percentage difference from the 1901–1960 mean. The annual values are averaged over 5-year periods, with the pentad label indicating the ending year of the period. Annual time series of the number of events are first calculated at individual stations. Next, the grid box time series are calculated as the average of all stations in the grid box. Finally, a national time series is calculated as the average of the grid box time series. Data source: GHCN-Daily. (Figure source: CICS-NC and NOAA NCEI).
Maximum daily precipitation totals were calculated for consecutive 5-year blocks from 1901 (1901–1905, 1906–1910, 1911–1915, …, 2011–2016) for individual long-term stations. For each 5-year block, these values were aggregated to the regional scale by first arithmetically averaging the station 5-year maximum for all stations within each 2° by 2° latitude/longitude grid and then averaging across all grids within each region to create a regional time series. Finally, a trend was computed for the resulting regional time series. The difference between these two periods (Figure 7.4, upper left panel) indicates substantial increases over the eastern United States, particularly the northeastern United States with an increase of 27% since 1901. The increases are much smaller over the western United States, with the southwestern and northwestern United States showing little increase.
Another index of extreme precipitation from NCA3 (the total precipitation falling in the top 1% of all days with precipitation) was updated through 2016 (Figure 7.4, upper right panel). This analysis is for 1958–2016. There are increases in all regions, with the largest increases again in the northeastern United States. There are some changes in the values compared to NCA3, with small increases in some regions such as the Midwest and Southwest and small decreases in others such as the Northeast, but the overall picture of changes is the same.
The national results shown in Figure 7.3 were disaggregated into regional values for two periods: 1901–2016 (Figure 7.4, lower left panel) and 1958–2016 (Figure 7.4, lower right panel) for comparison with Figure 7.4, upper right panel. As with the other metrics, there are large increases over the eastern half of the United States while the increases in the western United States are smaller and there are actually small decreases in the Southwest.
There are differences in the magnitude of changes among the four different regional metrics in Figure 7.4, but the overall picture is the same: large increases in the eastern half of the United States and smaller increases, or slight decreases, in the western United States.
Figure 7.4
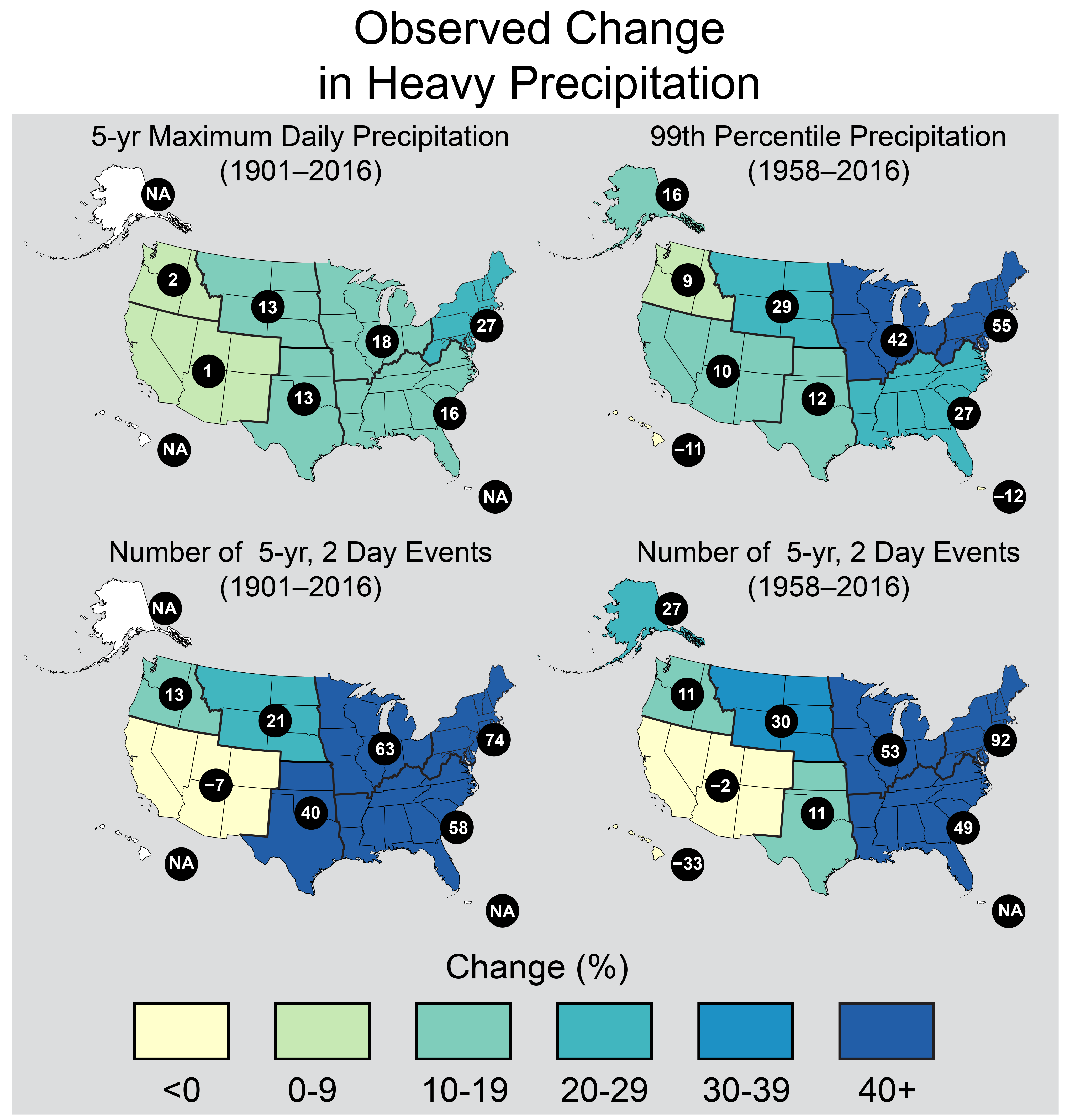
These maps show the change in several metrics of extreme precipitation by NCA4 region, including (upper left) the maximum daily precipitation in consecutive 5-year blocks, (upper right) the amount of precipitation falling in daily events that exceed the 99th percentile of all non-zero precipitation days, (lower left) the number of 2-day events with a precipitation total exceeding the largest 2-day amount that is expected to occur, on average, only once every 5 years, as calculated over 1901–2016, and (lower right) the number of 2-day events with a precipitation total exceeding the largest 2-day amount that is expected to occur, on average, only once every 5 years, as calculated over 1958–2016. The numerical value is the percent change over the entire period, either 1901–2016 or 1958–2016. The percentages are first calculated for individual stations, then averaged over 2° latitude by 2° longitude grid boxes, and finally averaged over each NCA4 region. Note that Alaska and Hawai‘i are not included in the 1901–2016 maps owing to a lack of observations in the earlier part of the 20th century. (Figure source: CICS-NC and NOAA NCEI).
7.1.4 Extratropical Cyclones and Mesoscale Convective Systems
As described in Chapter 9: Extreme Storms, there is uncertainty about future changes in winter extratropical cyclones (ETCs).19 Thus, the potential effects on winter extreme precipitation events is also uncertain. Summertime ETC activity across North America has decreased since 1979, with a reduction of more than 35% in the number of strong summertime ETCs.20 Most climate models simulate little change over this same historical period, but they project a decrease in summer ETC activity during the remainder of the 21st century.20 This is potentially relevant to extreme precipitation in the northeastern quadrant of the United States because a large percentage of the extreme precipitation events in this region are caused by ETCs and their associated fronts.21 This suggests that in the future there may be fewer opportunities in the summer for extreme precipitation, although increases in water vapor are likely to overcompensate for any decreases in ETCs by increasing the likelihood that an ETC will produce excessive rainfall amounts. A very idealized set of climate simulations22 suggests that substantial projected warming will lead to a decrease in the number of ETCs but an increase in the intensity of the strongest ETCs. One factor potentially causing this model ETC intensification is an increase in latent heat release in these storms related to a moister atmosphere. Because of the idealized nature of these simulations, the implications of these results for the real earth–atmosphere system is uncertain. However, the increased latent heat mechanism is likely to occur given the high confidence in a future moister atmosphere. For eastern North America, CMIP5 simulations of the future indicate an increase in strong ETCs.19 Thus, it is possible that the most extreme precipitation events associated with ETCs may increase in the future.
Mesoscale convective systems (MCSs), which contribute substantially to warm season precipitation in the tropics and subtropics,23 account for about half of rainfall in the central United States.24 Schumacher and Johnson25 reported that 74% of all warm season extreme rain events over the eastern two-thirds of the United States during the period 1999–2003 were associated with an MCS. Feng et al.26 found that large regions of the central United States experienced statistically significant upward trends in April–June MCS rainfall of 0.4–0.8 mm per day (approximately 20%–40%) per decade from 1979 to 2014. They further found upward trends in MCS frequency of occurrence, lifetime, and precipitation amount, which they attribute to an enhanced west-to-east pressure gradient (enhanced Great Plains low-level jet) and enhanced specific humidity throughout the eastern Great Plains.
7.1.5 Detection and Attribution
TRENDS
Detectability of trends (compared to internal variability) for a number of precipitation metrics over the continental United States has been examined; however, trends identified for the U.S. regions have not been clearly attributed to anthropogenic forcing.27 ,28 One study concluded that increasing precipitation trends in some north-central U.S. regions and the extreme annual anomalies there in 2013 were at least partly attributable to the combination of anthropogenic and natural forcing.29
There is medium confidence that anthropogenic forcing has contributed to global-scale intensification of heavy precipitation over land regions with sufficient data coverage.30 Global changes in extreme precipitation have been attributed to anthropogenically forced climate change,31 ,32 including annual maximum 1-day and 5-day accumulated precipitation over Northern Hemisphere land regions and (relevant to this report) over the North American continent.33 Although the United States was not separately assessed, the parts of North America with sufficient data for analysis included the continental United States and parts of southern Canada, Mexico, and Central America. Since the covered region was predominantly over the United States, these detection/attribution findings are applicable to the continental United States.
Analyses of precipitation extreme changes over the United States by region (20-year return values of seasonal daily precipitation over 1948–2015, Figure 7.2) show statistically significant increases consistent with theoretical expectations and previous analyses.34 Further, a significant increase in the area affected by precipitation extremes over North America has also been detected.35 There is likely an anthropogenic influence on the upward trend in heavy precipitation,36 although models underestimate the magnitude of the trend. Extreme rainfall from U.S. landfalling tropical cyclones has been higher in recent years (1994–2008) than the long-term historical average, even accounting for temporal changes in storm frequency.10
Based on current evidence, it is concluded that detectable but not attributable increases in mean precipitation have occurred over parts of the central United States. Formal detection-attribution studies indicate a human contribution to extreme precipitation increases over the continental United States, but confidence is low based on those studies alone due to the short observational period, high natural variability, and model uncertainty.
In summary, based on available studies, it is concluded that for the continental United States there is high confidence in the detection of extreme precipitation increases, while there is low confidence in attributing the extreme precipitation changes purely to anthropogenic forcing. There is stronger evidence for a human contribution (medium confidence) when taking into account process-based understanding (increased water vapor in a warmer atmosphere), evidence from weather and climate models, and trends in other parts of the world.
EVENT ATTRIBUTION
A number of recent heavy precipitation events have been examined to determine the degree to which their occurrence and severity can be attributed to human-induced climate change. Table 7.1 summarizes available attribution statements for recent extreme U.S. precipitation events. Seasonal and annual precipitation extremes occurring in the north-central and eastern U.S. regions in 2013 were examined for evidence of an anthropogenic influence on their occurrence.29 Increasing trends in annual precipitation were detected in the northern tier of states, March–May precipitation in the upper Midwest, and June–August precipitation in the eastern United States since 1900. These trends are attributed to external forcing (anthropogenic and natural) but could not be directly attributed to anthropogenic forcing alone. However, based on this analysis, it is concluded that the probability of these kinds of extremes has increased due to anthropogenic forcing.
The human influence on individual storms has been investigated with conflicting results. For example, in examining the attribution of the 2013 Colorado floods, one study finds that despite the expected human-induced increase in available moisture, the GEOS-5 model produces fewer extreme storms in the 1983–2012 period compared to the 1871–1900 period in Colorado during the fall season; the study attributes that behavior to changes in the large-scale circulation.37 However, another study finds that such coarse models cannot produce the observed magnitude of precipitation due to resolution constraints.38 Based on a highly conditional set of hindcast simulations imposing the large-scale meteorology and a substantial increase in both the probability and magnitude of the observed precipitation accumulation magnitudes in that particular meteorological situation, the study could not address the question of whether such situations have become more or less probable. Extreme precipitation event attribution is inherently limited by the rarity of the necessary meteorological conditions and the limited number of model simulations that can be performed to examine rare events. This remains an open and active area of research. However, based on these two studies, the anthropogenic contribution to the 2013 Colorado heavy rainfall-flood event is unclear.
An event attribution study of the potential influence of anthropogenic climate change on the extreme 3-day rainfall event associated with flooding in Louisiana in August 201639 finds that such extreme rainfall events have become more likely since 1900. Model simulations of extreme rainfall suggest that anthropogenic forcing has increased the odds of such a 3-day extreme precipitation event by 40% or more.
Authors | Event year and duration | Region | Type | Attribution statement |
---|---|---|---|---|
Knutson et al. 201429 / Angélil et al. 201776 | ANN 2013 | U.S. Northern Tier | Wet | +/0 |
Knutson et al. 201429 / Angélil et al. 201776 | MAM 2013 | U.S. Upper Midwest | Wet | +/+ |
Knutson et al. 201429 / Angélil et al. 201776 | JJA 2013 | Eastern U.S. Region | Wet | +/- |
Edwards et al. 201477 | October 4-5, 2013 | South Dakota | Blizzard | 0 |
Hoerling et al. 201437 | September 10-14, 2013 | Colorado | Wet | 0 |
Pall et al. 201738 | September 10-14, 2013 | Colorado | Wet | + |
7.2: Projections
Changes in precipitation in a warmer climate are governed by many factors. Although energy constraints can be used to understand global changes in precipitation, projecting regional changes is much more difficult because of uncertainty in projecting changes in the large-scale circulation that plays an important role in the formation of clouds and precipitation.40 For the contiguous United States (CONUS), future changes in seasonal average precipitation will include a mix of increases, decreases, or little change, depending on location and season (Figure 7.5). High-latitude regions are generally projected to become wetter while the subtropical zone is projected to become drier. As the CONUS lies between these two regions, there is significant uncertainty about the sign and magnitude of future anthropogenic changes to seasonal precipitation in much of the region, particularly in the middle latitudes of the Nation. However, because the physical mechanisms controlling extreme precipitation differ from those controlling seasonal average precipitation (Section 7.1.4), in particular atmospheric water vapor will increase with increasing temperatures, confidence is high that precipitation extremes will increase in frequency and intensity in the future throughout the CONUS.
Global climate models used to project precipitation changes exhibit varying degrees of fidelity in capturing the observed climatology and seasonal variations of precipitation across the United States. Global or regional climate models with higher horizontal resolution generally achieve better skill than the CMIP5 models in capturing the spatial patterns and magnitude of winter precipitation in the western and southeastern United States (e.g., Mearns et al. 2012;41 Wehner 2013;42 Bacmeister et al. 2014;43 Wehner et al. 201444 ), leading to improved simulations of snowpack and runoff (e.g., Rauscher et al. 2008;45 Rasmussen et al. 201146 ). Simulation of present and future summer precipitation remains a significant challenge, as current convective parameterizations fail to properly represent the statistics of mesoscale convective systems.47 As a result, high-resolution models that still require the parameterization of deep convection exhibit mixed results.44 ,48 Advances in computing technology are beginning to enable regional climate modeling at the higher resolutions (1–4 km), permitting the direct simulation of convective clouds systems (e.g., Ban et al. 201449 ) and eliminating the need for this class of parameterization. However, projections from such models are not yet ready for inclusion in this report.
Important progress has been made by the climate modeling community in providing multimodel ensembles such as CMIP550 and NARCCAP41 to characterize projection uncertainty arising from model differences and large ensemble simulations such as CESM-LE51 to characterize uncertainty inherent in the climate system due to internal variability. These ensembles provide an important resource for examining the uncertainties in future precipitation projections.
7.2.1 Future Changes in U.S. Seasonal Mean Precipitation
In the United States, projected changes in seasonal mean precipitation span the range from profound decreases to profound increases. In many regions and seasons, projected changes in precipitation are not large compared to natural variations. The general pattern of change is clear and consistent with theoretical expectations. Figure 7.5 shows the weighted CMIP5 multimodel average seasonal change at the end of the century compared to the present under the higher scenario (RCP8.5; see Ch. 4: Projections for discussion of RCPs). In this figure, changes projected with high confidence to be larger than natural variations are stippled. Regions where future changes are projected with high confidence to be smaller than natural variations are hatched. In winter and spring, the northern part of the country is projected to become wetter as the global climate warms. In the early to middle parts of this century, this will likely be manifested as increases in snowfall.52 By the latter half of the century, as temperature continues to increase, it will be too warm to snow in many current snow-producing situations, and precipitation will mostly be rainfall. In the southwestern United States, precipitation will decrease in the spring but the changes are only a little larger than natural variations. Many other regions of the country will not experience significant changes in average precipitation. This is also the case over most of the country in the summer and fall.
Figure 7.5
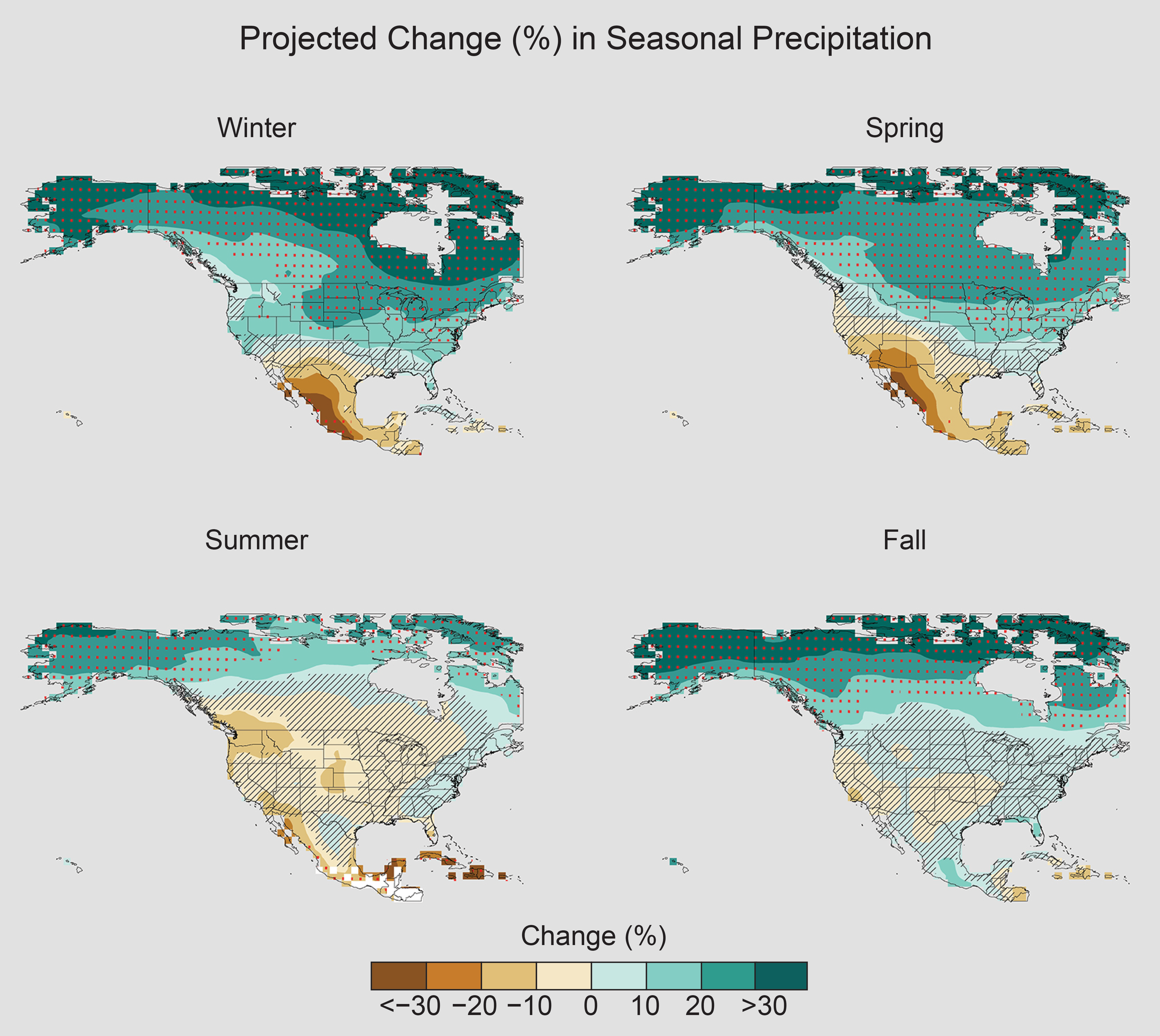
Projected change (%) in total seasonal precipitation from CMIP5 simulations for 2070–2099. The values are weighted multimodel means and expressed as the percent change relative to the 1976–2005 average. These are results for the higher scenario (RCP8.5). Stippling indicates that changes are assessed to be large compared to natural variations. Hatching indicates that changes are assessed to be small compared to natural variations. Blank regions (if any) are where projections are assessed to be inconclusive. Data source: World Climate Research Program’s (WCRP’s) Coupled Model Intercomparison Project. (Figure source: NOAA NCEI).
This pattern of projected precipitation change arises because of changes in locally available water vapor and weather system shifts. In the northern part of the continent, increases in water vapor, together with changes in circulation that are the result of expansion of the Hadley cell, bring more moisture to these latitudes while maintaining or increasing the frequency of precipitation-producing weather systems. This change in the Hadley circulation (see Ch. 5: Circulation and Variability for discussion of circulation changes) also causes the subtropics, the region between the northern and southern edges of the tropics and the midlatitudes (about 35° of latitude), to be drier in warmer climates as well as moving the mean storm track northward and away from the subtropics, decreasing the frequency of precipitation-producing systems. The combination of these two factors results in precipitation decreases in the southwestern United States, Mexico, and the Caribbean.53
PROJECTED CHANGES IN SNOW
The Third National Climate Assessment54 projected reductions in annual snowpack of up to 40% in the western United States based on the SRES A2 emissions scenario in the CMIP3 suite of climate model projections. Recent research using the CMIP5 suite of climate model projections forced with a higher scenario (RCP8.5) and statistically downscaled for the western United States continues to show the expected declines in various snow metrics, including snow water equivalent, the number of extreme snowfall events, and number of snowfall days.55 A northward shift in the rain–snow transition zone in the central and eastern United States was found using statistically downscaled CMIP5 simulations forced with RCP8.5. By the end of the 21st century, large areas that are currently snow dominated in the cold season are expected to be rainfall dominated.56
The Variable Infiltration Capacity (VIC) model has been used to investigate the potential effects of climate change on SWE. Declines in SWE are projected in all western U.S. mountain ranges during the 21st century with the virtual disappearance of snowpack in the southernmost mountains by the end of the 21st century under both the lower (RCP4.5) and higher (RCP8.5) scenarios.57 The projected decreases are most robust at the lower elevations of areas where snowpack accumulation is now reliable (for example, the Cascades and northern Sierra Nevada ranges). In these areas, future decreases in SWE are largely driven by increases in temperature. At higher (colder) elevations, projections are driven more by precipitation changes and are thus more uncertain.
7.2.2 Extremes
HEAVY PRECIPITATION EVENTS
Studies project that the observed increase in heavy precipitation events will continue in the future (e.g. Janssen et al. 2014,58 201659 ). Similar to observed changes, increases are expected in all regions, even those regions where total precipitation is projected to decline, such as the southwestern United States. Under the higher scenario (RCP8.5) the number of extreme events (exceeding a 5-year return period) increases by two to three times the historical average in every region (Figure 7.6) by the end of the 21st century, with the largest increases in the Northeast. Under the lower scenario (RCP4.5), increases are 50%–100%. Research shows that there is strong evidence, both from the observed record and modeling studies, that increased water vapor resulting from higher temperatures is the primary cause of the increases.42 ,60 ,61 Additional effects on extreme precipitation due to changes in dynamical processes are poorly understood. However, atmospheric rivers (ARs), especially along the West Coast of the United States, are projected to increase in number and water vapor transport62 and experience landfall at lower latitudes63 by the end of the 21st century.
Figure 7.6
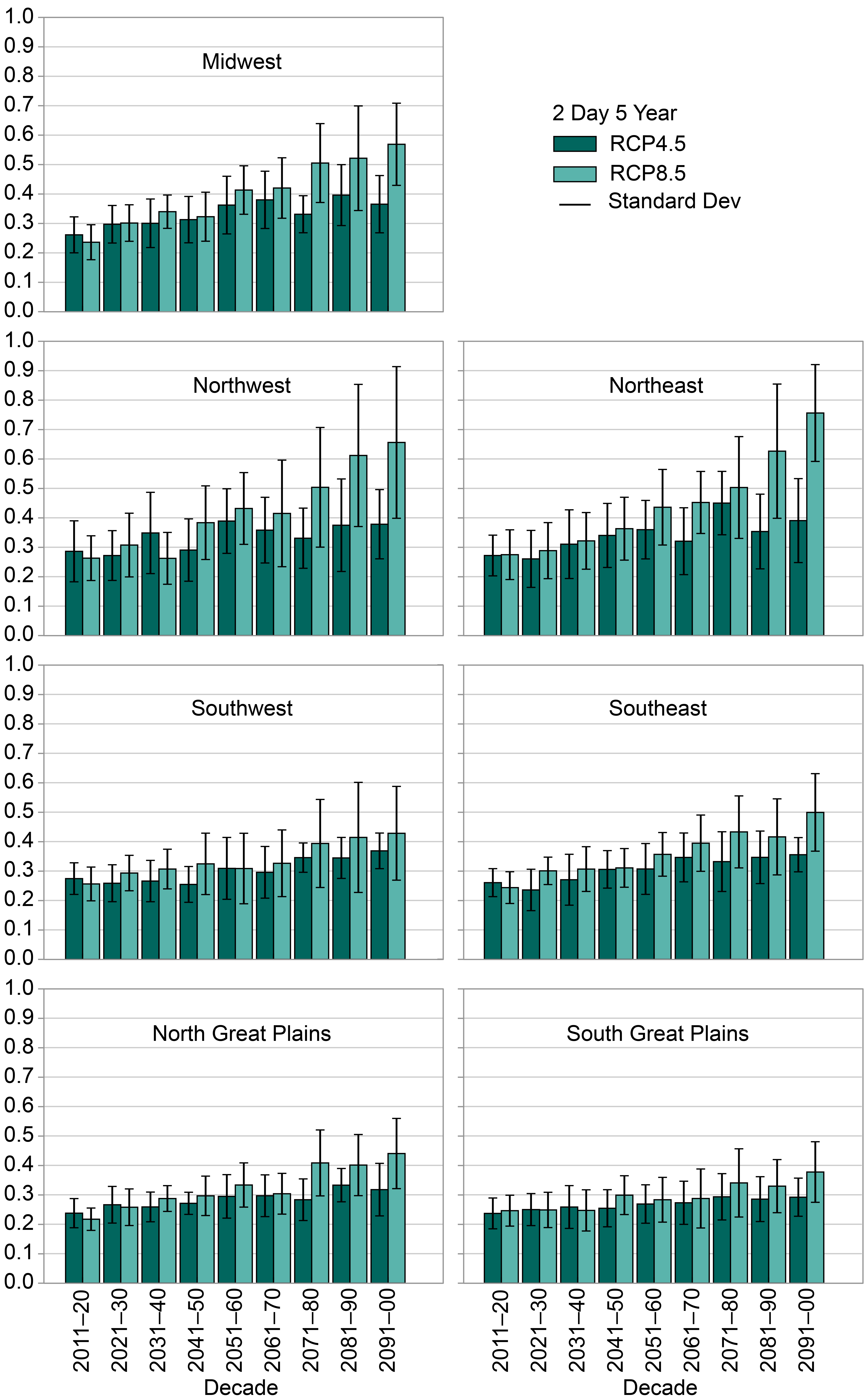
Regional extreme precipitation event frequency for a lower scenario (RCP4.5) (green; 16 CMIP5 models) and the higher scenario (RCP8.5) (blue; 14 CMIP5 models) for a 2-day duration and 5-year return. Calculated for 2006–2100 but decadal anomalies begin in 2011. Error bars are ±1 standard deviation; standard deviation is calculated from the 14 or 16 model values that represent the aggregated average over the regions, over the decades, and over the ensemble members of each model. The average frequency for the historical reference period is 0.2 by definition and the values in this graph should be interpreted with respect to a comparison with this historical average value. (Figure source: Janssen et al. 201458 ).
Projections of changes in the 20-year return period amount for daily precipitation (Figure 7.7) using LOcally Constructed Analogs (LOCA) downscaled data also show large percentage increases for both the middle and late 21st century. A lower scenario (RCP4.5) show increases of around 10% for mid-century and up to 14% for the late century projections. A higher scenario (RCP8.5) shows even larger increases for both mid- and late-century projections, with increases of around 20% by late 21st century. No region in either scenario shows a decline in heavy precipitation. The increases in extreme precipitation tend to increase with return level, such that increases for the 100-year return level are about 30% by the end of the century under a higher scenario (RCP8.5).
Figure 7.7
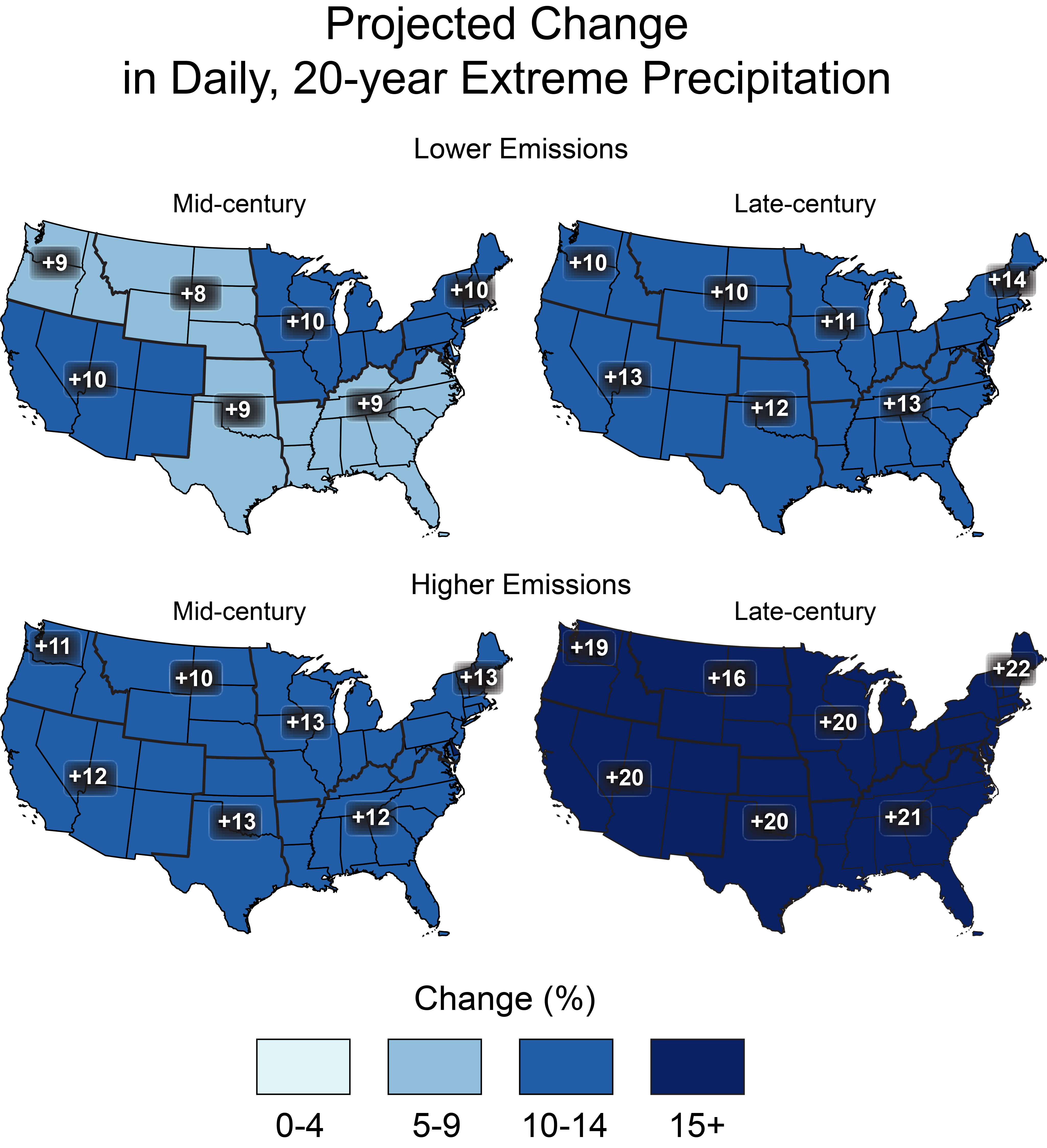
Projected change in the 20-year return period amount for daily precipitation for mid- (left maps) and late-21st century (right maps). Results are shown for a lower scenario (top maps; RCP4.5) and for a higher scenario (bottom maps, RCP8.5). These results are calculated from the LOCA downscaled data. (Figure source: CICS-NC and NOAA NCEI).
Projections of changes in the distribution of daily precipitation amounts (Figure 7.8) indicate an overall more extreme precipitation climate. Specifically, the projections indicate a slight increase in the numbers of dry days and the very lightest precipitation days and a large increase in the heaviest days. The number of days with precipitation amounts greater than the 95th percentile of all non-zero precipitation days increases by more than 25%. At the same time, the number of days with precipitation amounts in the 10th–80th percentile range decreases.
Figure 7.8
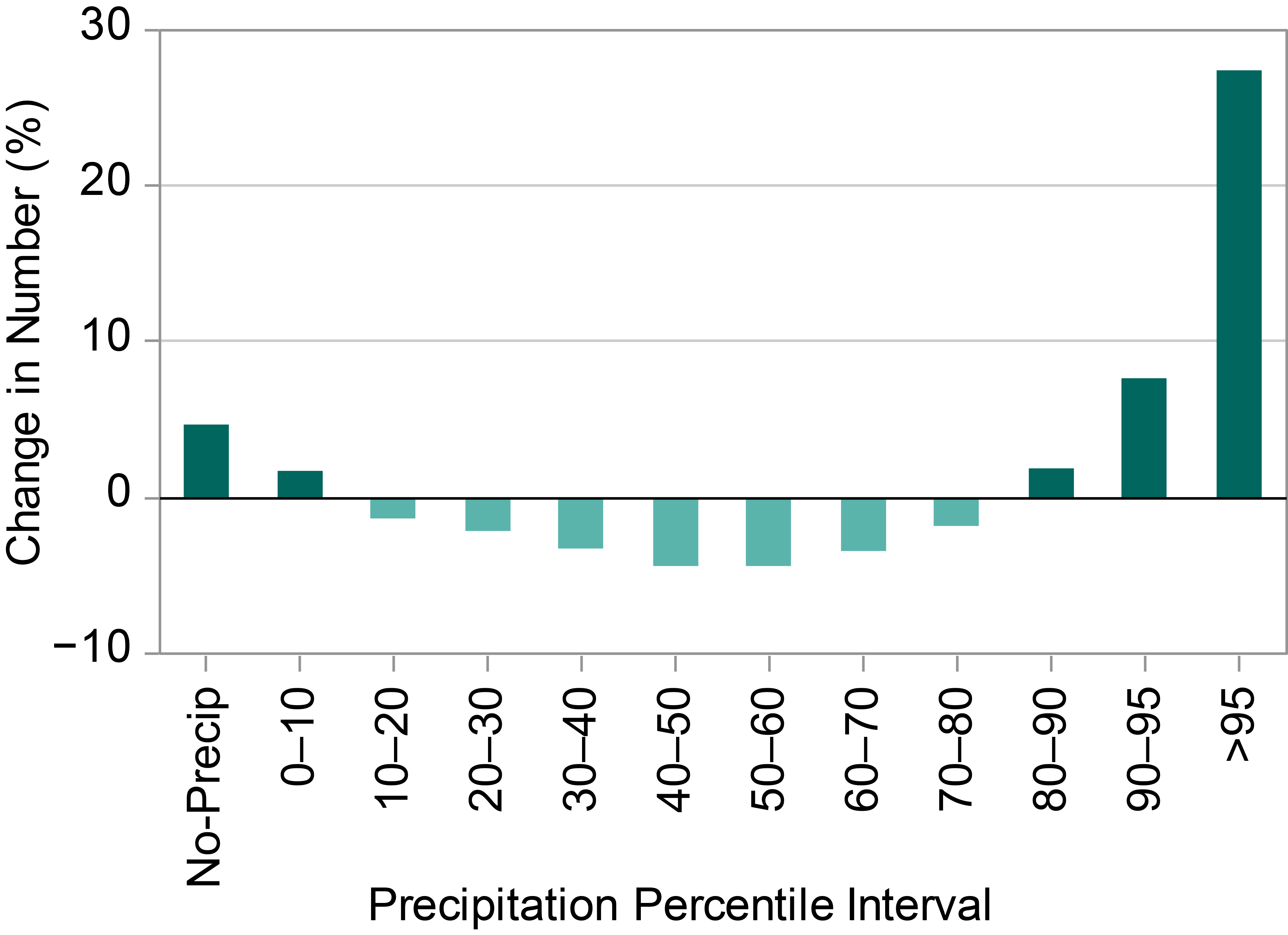
Projected change (percentage change relative to the 1976–2005 reference period average) in the number of daily zero (“No-Precip”) and non-zero precipitation days (by percentile bins) for late-21st century under a higher scenario (RCP8.5). The precipitation percentile bin thresholds are based on daily non-zero precipitation amounts from the 1976–2005 reference period that have been ranked from low to high. These results are calculated from the LOCA downscaled data. (Figure source: CICS-NC and NOAA NCEI).
Most global climate models lack sufficient resolution to project changes in mesoscale convective systems (MCSs) in a changing climate.64 However, research by Cook et al.65 attempted to identify clues to changes in dynamical forcing that create MCSs. To do this, they examined the ability of 18 coupled ocean–atmosphere global climate models (GCMs) to simulate potential 21st century changes in warm-season flow and the associated U.S. Midwest hydrology resulting from increases in greenhouse gases. They selected a subset of six models that best captured the low-level flow and associated dynamics of the present-day climate of the central United States and then analyzed these models for changes due to enhanced greenhouse gas forcing. In each of these models, springtime precipitation increases significantly (by 20%–40%) in the upper Mississippi Valley and decreases to the south. The enhanced moisture convergence leading to modeled future climate rainfall increases in the U.S. Midwest is caused by meridional convergence at 850 hPa, connecting the rainfall changes with the Great Plains Low-Level Jet intensification.66 This is consistent with findings from Feng et al.26 in the observational record for the period 1979–2014 and by Pan et al.67 by use of a regional climate model.
Changes in intense hourly precipitation events were simulated by Prein et al.68 where they found the most intense hourly events (99.9 percentile) in the central United States increase at the expense of moderately intense (97.5 percentile) hourly events in the warm season. They also found the frequency of seasonal hourly precipitation extremes is expected to increase in all regions by up to five times in the same areas that show the highest increases in extreme precipitation rates.
HURRICANE PRECIPITATION
Regional model projections of precipitation from landfalling tropical cyclones over the United States, based on downscaling of CMIP5 model climate changes, suggest that the occurrence frequency of post-landfall tropical cyclones over the United States will change little compared to present day during the 21st century, as the reduced frequency of tropical cyclones over the Atlantic domain is mostly offset by a greater landfalling fraction. However, when downscaling from CMIP3 model climate changes, projections show a reduced occurrence frequency over U.S. land, indicating uncertainty about future outcomes. The average tropical cyclone rainfall rates within 500 km (about 311 miles) of the storm center increased by 8% to 17% in the simulations, which was at least as much as expected from the water vapor content increase factor alone.
Several studies have projected increases of precipitation rates within hurricanes over ocean regions,69 particularly for the Atlantic basin.70 The primary physical mechanism for this increase is the enhanced water vapor content in the warmer atmosphere, which enhances moisture convergence into the storm for a given circulation strength, although a more intense circulation can also contribute.71 Since hurricanes are responsible for many of the most extreme precipitation events in the southeastern United States,10 ,21 such events are likely to be even heavier in the future. In a set of idealized forcing experiments, this effect was partly offset by differences in warming rates at the surface and at altitude.72
References
- Anderson, B. T., D. J. Gianotti, and G. D. Salvucci, 2015: Detectability of historical trends in station-based precipitation characteristics over the continental United States. Journal of Geophysical Research Atmospheres, 120, 4842–4859, doi:10.1002/2014JD022960. ↩
- Angélil, O., D. Stone, M. Wehner, C. J. Paciorek, H. Krishnan, and W. Collins, 2017: An independent assessment of anthropogenic attribution statements for recent extreme temperature and rainfall events. Journal of Climate, 30, 5–16, doi:10.1175/JCLI-D-16-0077.1. ↩
- Bacmeister, J. T., M. F. Wehner, R. B. Neale, A. Gettelman, C. Hannay, P. H. Lauritzen, J. M. Caron, and J. E. Truesdale, 2014: Exploratory high-resolution climate simulations using the Community Atmosphere Model (CAM). Journal of Climate, 27, 3073–3099, doi:10.1175/JCLI-D-13-00387.1. ↩
- Ban, N., J. Schmidli, and C. Schär, 2014: Evaluation of the convection-resolving regional climate modeling approach in decade-long simulations. Journal of Geophysical Research Atmospheres, 119, 7889–7907, doi:10.1002/2014JD021478. ↩
- Bard, L., and D. A. R. Kristovich, 2012: Trend reversal in Lake Michigan contribution to snowfall. Journal of Applied Meteorology and Climatology, 51, 2038–2046, doi:10.1175/jamc-d-12-064.1. ↩
- Barnston, A. G., and B. Lyon, 2016: Does the NMME capture a recent decadal shift toward increasing drought occurrence in the southwestern United States? Journal of Climate, 29, 561–581, doi:10.1175/JCLI-D-15-0311.1. ↩
- Belmecheri, S., F. Babst, E. R. Wahl, D. W. Stahle, and V. Trouet, 2016: Multi-century evaluation of Sierra Nevada snowpack. Nature Climate Change, 6, 2–3, doi:10.1038/nclimate2809. ↩
- Bindoff, N. L., P. A. Stott, K. M. AchutaRao, M. R. Allen, N. Gillett, D. Gutzler, K. Hansingo, G. Hegerl, Y. Hu, S. Jain, I. I. Mokhov, J. Overland, J. Perlwitz, R. Sebbari, and X. Zhang, 2013: Detection and attribution of climate change: From global to regional. T.F. Stocker, D. Qin, G.-K. Plattner, M. Tignor, S.K. Allen, J. Boschung, A. Nauels, Y. Xia, V. Bex, and P.M. Midgley, Eds., Cambridge University Press, 867–952. URL ↩
- Boyle, J., and S. A. Klein, 2010: Impact of horizontal resolution on climate model forecasts of tropical precipitation and diabatic heating for the TWP-ICE period. Journal of Geophysical Research, 115, D23113, doi:10.1029/2010JD014262. ↩
- Chang, E. K. M., C.-G. Ma, C. Zheng, and A. M. W. Yau, 2016: Observed and projected decrease in Northern Hemisphere extratropical cyclone activity in summer and its impacts on maximum temperature. Geophysical Research Letters, 43, 2200–2208, doi:10.1002/2016GL068172. ↩
- Colle, B. A., Z. Zhang, K. A. Lombardo, E. Chang, P. Liu, and M. Zhang, 2013: Historical evaluation and future prediction of eastern North American and western Atlantic extratropical cyclones in the CMIP5 models during the cool season. Journal of Climate, 26, 6882–6903, doi:10.1175/JCLI-D-12-00498.1. ↩
- Collins, M., R. Knutti, J. Arblaster, J.-L. Dufresne, T. Fichefet, P. Friedlingstein, X. Gao, W. J. Gutowski, T. Johns, G. Krinner, M. Shongwe, C. Tebaldi, A. J. Weaver, and M. Wehner, 2013: Long-term climate change: Projections, commitments and irreversibility. T.F. Stocker, D. Qin, G.-K. Plattner, M. Tignor, S.K. Allen, J. Boschung, A. Nauels, Y. Xia, V. Bex, and P.M. Midgley, Eds., Cambridge University Press, 1029–1136. URL ↩
- Cook, K. H., E. K. Vizy, Z. S. Launer, and C. M. Patricola, 2008: Springtime intensification of the Great Plains low-level jet and midwest precipitation in GCM simulations of the twenty-first century. Journal of Climate, 21, 6321–6340, doi:10.1175/2008jcli2355.1. ↩
- Dettinger, M., 2011: Climate change, atmospheric rivers, and floods in California–a multimodel analysis of storm frequency and magnitude changes. Journal of the American Water Resources Association, 47, 514–523, doi:10.1111/j.1752-1688.2011.00546.x. ↩
- Dittus, A. J., D. J. Karoly, S. C. Lewis, L. V. Alexander, and M. G. Donat, 2016: A multiregion model evaluation and attribution study of historical changes in the area affected by temperature and precipitation extremes. Journal of Climate, 29, 8285–8299, doi:10.1175/jcli-d-16-0164.1. ↩
- Dittus, A. J., D. J. Karoly, S. C. Lewis, and L. V. Alexander, 2015: A multiregion assessment of observed changes in the areal extent of temperature and precipitation extremes. Journal of Climate, 28, 9206–9220, doi:10.1175/JCLI-D-14-00753.1. ↩
- Easterling, D. R., K. E. Kunkel, M. F. Wehner, and L. Sun, 2016: Detection and attribution of climate extremes in the observed record. Weather and Climate Extremes, 11, 17–27, doi:10.1016/j.wace.2016.01.001. ↩
- Edwards, L. M., M. . Bunkers, J. T. Abatzoglou, D. P. Todey, and L. E. Parker, 2014: October 2013 blizzard in western South Dakota [in “Explaining Extreme Events of 2013 from a Climate Perspective”]. Bulletin of the American Meteorological Society, 95 (9), S23–S26, doi:10.1175/1520-0477-95.9.S1.1. ↩
- Feng, Z., L. R. Leung, S. Hagos, R. A. Houze, C. D. Burleyson, and K. Balaguru, 2016: More frequent intense and long-lived storms dominate the springtime trend in central US rainfall. Nature Communications, 7, 13429, doi:10.1038/ncomms13429. ↩
- Fritsch, J. M., R. J. Kane, and C. R. Chelius, 1986: The contribution of mesoscale convective weather systems to the warm-season precipitation in the United States. Journal of Climate and Applied Meteorology, 25, 1333–1345, doi:10.1175/1520-0450(1986)025<1333:tcomcw>2.0.co;2. ↩
- Gan, T. Y., R. G. Barry, M. Gizaw, A. Gobena, and R. Balaji, 2013: Changes in North American snowpacks for 1979–2007 detected from the snow water equivalent data of SMMR and SSM/I passive microwave and related climatic factors. Journal of Geophysical Research: Atmospheres, 118, 7682–7697, doi:10.1002/jgrd.50507. ↩
- Georgakakos, A., P. Fleming, M. Dettinger, C. Peters-Lidard, Terese (T.C.) Richmond, K. Reckhow, K. White, and D. Yates, 2014: Ch. 3: Water Resources. J.M. Melillo, T. (T. C.. Richmond, and G.W. Yohe, Eds., U.S. Global Change Research Program, 69–112. ↩
- Gergel, D. R., B. Nijssen, J. T. Abatzoglou, D. P. Lettenmaier, and M. R. Stumbaugh, 2017: Effects of climate change on snowpack and fire potential in the western USA. Climatic Change, 141, 287–299, doi:10.1007/s10584-017-1899-y. ↩
- Hartnett, J. J., J. M. Collins, M. A. Baxter, and D. P. Chambers, 2014: Spatiotemporal snowfall trends in central New York. Journal of Applied Meteorology and Climatology, 53, 2685–2697, doi:10.1175/jamc-d-14-0084.1. ↩
- Higgins, R. W., Y. Yao, E. S. Yarosh, J. E. Janowiak, and K. C. Mo, 1997: Influence of the Great Plains low-level jet on summertime precipitation and moisture transport over the central United States. Journal of Climate, 10, 481–507, doi:10.1175/1520-0442(1997)010<0481:iotgpl>2.0.co;2. ↩
- Hoerling, M., K. Wolter, J. Perlwitz, X. Quan, J. Eischeid, H. Want, S. Schubert, H. Diaz, and R. Dole, 2014: Northeast Colorado extreme rains interpreted in a climate change context [in “Explaining Extreme Events of 2013 from a Climate Perspective”]. Bulletin of the American Meteorological Society, 95 (9), S15–S18, doi:10.1175/1520-0477-95.9.S1.1. ↩
- Janssen, E., D. J. Wuebbles, K. E. Kunkel, S. C. Olsen, and A. Goodman, 2014: Observational- and model-based trends and projections of extreme precipitation over the contiguous United States. Earth’s Future, 2, 99–113, doi:10.1002/2013EF000185. ↩
- Janssen, E., R. L. Sriver, D. J. Wuebbles, and K. E. Kunkel, 2016: Seasonal and regional variations in extreme precipitation event frequency using CMIP5. Geophysical Research Letters, 43, 5385–5393, doi:10.1002/2016GL069151. ↩
- Kay, J. E. et al., 2015: The Community Earth System Model (CESM) large ensemble project: A community resource for studying climate change in the presence of internal climate variability. Bulletin of the American Meteorological Society, 96 (12), 1333–1349, doi:10.1175/BAMS-D-13-00255.1. ↩
- Kluver, D., and D. Leathers, 2015: Regionalization of snowfall frequency and trends over the contiguous United States. International Journal of Climatology, 35, 4348–4358, doi:10.1002/joc.4292. ↩
- Knutson, T. R., F. Zeng, and A. T. Wittenberg, 2014: Seasonal and annual mean precipitation extremes occurring during 2013: A U.S. focused analysis [in “Explaining Extreme Events of 2013 from a Climate Perspective”]. Bulletin of the American Meteorological Society, 95 (9), S19–S23, doi:10.1175/1520-0477-95.9.S1.1. ↩
- Knutson, T. R., J. J. Sirutis, G. A. Vecchi, S. Garner, M. Zhao, H.-S. Kim, M. Bender, R. E. Tuleya, I. M. Held, and G. Villarini, 2013: Dynamical downscaling projections of twenty-first-century Atlantic hurricane activity: CMIP3 and CMIP5 model-based scenarios. Journal of Climate, 27, 6591–6617, doi:10.1175/jcli-d-12-00539.1. ↩
- Knutson, T. R., J. L. McBride, J. Chan, K. Emanuel, G. Holland, C. Landsea, I. Held, J. P. Kossin, A. K. Srivastava, and M. Sugi, 2010: Tropical cyclones and climate change. Nature Geoscience, 3, 157–163, doi:10.1038/ngeo779. ↩
- Kooperman, G. J., M. S. Pritchard, and R. C. J. Somerville, 2013: Robustness and sensitivities of central U.S. summer convection in the super-parameterized CAM: Multi-model intercomparison with a new regional EOF index. Geophysical Research Letters, 40, 3287–3291, doi:10.1002/grl.50597. ↩
- Kunkel, K. E. et al., 2013: Monitoring and understanding trends in extreme storms: State of knowledge. Bulletin of the American Meteorological Society, 94, doi:10.1175/BAMS-D-11-00262.1. ↩
- Kunkel, K. E., D. A. Robinson, S. Champion, X. Yin, T. Estilow, and R. M. Frankson, 2016: Trends and extremes in Northern Hemisphere snow characteristics. Current Climate Change Reports, 2, 65–73, doi:10.1007/s40641-016-0036-8. ↩
- Kunkel, K. E., D. R. Easterling, D. A. Kristovich, B. Gleason, L. Stoecker, and R. Smith, 2012: Meteorological causes of the secular variations in observed extreme precipitation events for the conterminous United States. Journal of Hydrometeorology, 13, 1131–1141, doi:10.1175/JHM-D-11-0108.1. ↩
- Kunkel, K. E., D. R. Easterling, D. A. R. Kristovich, B. Gleason, L. Stoecker, and R. Smith, 2010: Recent increases in U.S. heavy precipitation associated with tropical cyclones. Geophysical Research Letters, 37, L24706, doi:10.1029/2010GL045164. ↩
- Kunkel, K. E., T. R. Karl, D. R. Easterling, K. Redmond, J. Young, X. Yin, and P. Hennon, 2013: Probable maximum precipitation and climate change. Geophysical Research Letters, 40, 1402–1408, doi:10.1002/grl.50334. ↩
- Luce, C. H., J. T. Abatzoglou, and Z. A. Holden, 2013: The missing mountain water: Slower westerlies decrease orographic enhancement in the Pacific Northwest USA. Science, 342, 1360–1364, doi:10.1126/science.1242335. ↩
- Lute, A. C., J. T. Abatzoglou, and K. C. Hegewisch, 2015: Projected changes in snowfall extremes and interannual variability of snowfall in the western United States. Water Resources Research, 51, 960–972, doi:10.1002/2014WR016267. ↩
- Lute, A. C., and J. T. Abatzoglou, 2014: Role of extreme snowfall events in interannual variability of snowfall accumulation in the western United States. Water Resources Research, 50, 2874–2888, doi:10.1002/2013WR014465. ↩
- Mearns, L. O. et al., 2012: The North American regional climate change assessment program: Overview of phase I results. Bulletin of the American Meteorological Society, 93, 1337–1362, doi:10.1175/BAMS-D-11-00223.1. ↩
- Min, S. K., X. Zhang, F. W. Zwiers, and G. C. Hegerl, 2011: Human contribution to more-intense precipitation extremes. Nature, 470, 378–381, doi:10.1038/nature09763. ↩
- Min, S.-K., X. Zhang, F. Zwiers, H. Shiogama, Y.-S. Tung, and M. Wehner, 2013: Multimodel detection and attribution of extreme temperature changes. Journal of Climate, 26, 7430–7451, doi:10.1175/JCLI-D-12-00551.1. ↩
- NOAA, 2016: Climate at a Glance: California PDSI. URL ↩
- NOAA, 2016: Climate at a Glance: Southwest PDSI. URL ↩
- Nesbitt, S. W., R. Cifelli, and S. A. Rutledge, 2006: Storm morphology and rainfall characteristics of TRMM precipitation features. Monthly Weather Review, 134, 2702–2721, doi:10.1175/mwr3200.1. ↩
- Ning, L., and R. S. Bradley, 2015: Snow occurrence changes over the central and eastern United States under future warming scenarios. Scientific Reports, 5, 17073, doi:10.1038/srep17073. ↩
- O’Gorman, P. A., 2014: Contrasting responses of mean and extreme snowfall to climate change. Nature, 512, 416–418, doi:10.1038/nature13625. ↩
- Pall, P. C. M. P., M. F. Wehner, D. A. Stone, C. J. Paciorek, and W. D. Collins, 2017: Diagnosing anthropogenic contributions to heavy Colorado rainfall in September 2013. Weather and Climate Extremes, 17, 1–6, doi:10.1016/j.wace.2017.03.004. ↩
- Pan, Z., R. W. Arritt, E. S. Takle, W. J. Gutowski Jr., C. J. Anderson, and M. Segal, 2004: Altered hydrologic feedback in a warming climate introduces a “warming hole.” Geophysical Research Letters, 31, L17109, doi:10.1029/2004GL020528. ↩
- Pederson, G. T., J. L. Betancourt, and G. J. McCabe, 2013: Regional patterns and proximal causes of the recent snowpack decline in the Rocky Mountains, U.S. Geophysical Research Letters, 40, 1811–1816, doi:10.1002/grl.50424. ↩
- Peterson, T. C. et al., 2013: Monitoring and understanding changes in heat waves, cold waves, floods and droughts in the United States: State of knowledge. Bulletin of the American Meteorological Society, 94, 821–834, doi:10.1175/BAMS-D-12-00066.1. ↩
- Pfahl, S., P. A. O’Gorman, and M. S. Singh, 2015: Extratropical cyclones in idealized simulations of changed climates. Journal of Climate, 28, 9373–9392, doi:10.1175/JCLI-D-14-00816.1. ↩
- Prein, A. F., R. M. Rasmussen, K. Ikeda, C. Liu, M. P. Clark, and G. J. Holland, 2017: The future intensification of hourly precipitation extremes. Nature Climate Change, 7, 48–52, doi:10.1038/nclimate3168. ↩
- Rasmussen, R., C. Liu, K. Ikeda, D. Gochis, D. Yates, F. Chen, M. Tewari, M. Barlage, J. Dudhia, W. Yu, K. Miller, K. Arsenault, V. Grubišić, G. Thompson, and E. Gutmann, 2011: High-resolution coupled climate runoff simulations of seasonal snowfall over Colorado: A process study of current and warmer climate. Journal of Climate, 24, 3015–3048, doi:10.1175/2010JCLI3985.1. ↩
- Rauscher, S. A., J. S. Pal, N. S. Diffenbaugh, and M. M. Benedetti, 2008: Future changes in snowmelt-driven runoff timing over the western US. Geophysical Research Letters, 35, L16703, doi:10.1029/2008GL034424. ↩
- Sakaguchi, K., L. R. Leung, C. Zhao, Q. Yang, J. Lu, S. Hagos, S. A. Rauscher, L. Dong, T. D. Ringler, and P. H. Lauritzen, 2015: Exploring a multiresolution approach using AMIP simulations. Journal of Climate, 28, 5549–5574, doi:10.1175/JCLI-D-14-00729.1. ↩
- Schumacher, R. S., and R. H. Johnson, 2006: Characteristics of U.S. extreme rain events during 1999–2003. Weather and Forecasting, 21, 69–85, doi:10.1175/waf900.1. ↩
- Seager, R., M. Hoerling, S. Schubert, H. Wang, B. Lyon, A. Kumar, J. Nakamura, and N. Henderson, 2015: Causes of the 2011–14 California drought. Journal of Climate, 28, 6997–7024, doi:10.1175/JCLI-D-14-00860.1. ↩
- Shepherd, T. G., 2014: Atmospheric circulation as a source of uncertainty in climate change projections. Nature Geoscience, 7, 703–708, doi:10.1038/ngeo2253. ↩
- Shields, C. A., and J. T. Kiehl, 2016: Atmospheric river landfall-latitude changes in future climate simulations. Geophysical Research Letters, 43, 8775–8782, doi:10.1002/2016GL070470. ↩
- Taylor, K. E., R. J. Stouffer, and G. A. Meehl, 2012: An overview of CMIP5 and the experiment design. Bulletin of the American Meteorological Society, 93, 485–498, doi:10.1175/BAMS-D-11-00094.1. ↩
- Vaughan, D. G., J. C. Comiso, I. Allison, J. Carrasco, G. Kaser, R. Kwok, P. Mote, T. Murray, F. Paul, J. Ren, E. Rignot, O. Solomina, K. Steffen, and T. Zhang, 2013: Observations: Cryosphere. T.F. Stocker, D. Qin, G.-K. Plattner, M. Tignor, S.K. Allen, J. Boschung, A. Nauels, Y. Xia, V. Bex, and P.M. Midgley, Eds., Cambridge University Press, 317–382. URL ↩
- Vavrus, S., M. Notaro, and A. Zarrin, 2013: The role of ice cover in heavy lake-effect snowstorms over the Great Lakes Basin as simulated by RegCM4. Monthly Weather Review, 141, 148–165, doi:10.1175/mwr-d-12-00107.1. ↩
- Villarini, G., D. A. Lavers, E. Scoccimarro, M. Zhao, M. F. Wehner, G. A. Vecchi, T. R. Knutson, and K. A. Reed, 2014: Sensitivity of tropical cyclone rainfall to idealized global-scale forcings. Journal of Climate, 27, 4622–4641, doi:10.1175/JCLI-D-13-00780.1. ↩
- Walsh, J. et al., 2014: Ch. 2: Our Changing Climate. J.M. Melillo, T. (T. C.. Richmond, and G.W. Yohe, Eds., U.S. Global Change Research Program, 19–67. ↩
- Wang, C.-C., B.-X. Lin, C.-T. Chen, and S.-H. Lo, 2015: Quantifying the effects of long-term climate change on tropical cyclone rainfall using a cloud-resolving model: Examples of two landfall typhoons in Taiwan. Journal of Climate, 28, 66–85, doi:10.1175/JCLI-D-14-00044.1. ↩
- Wehner, M. F., 2013: Very extreme seasonal precipitation in the NARCCAP ensemble: Model performance and projections. Climate Dynamics, 40, 59–80, doi:10.1007/s00382-012-1393-1. ↩
- Wehner, M. F., K. A. Reed, F. Li, Prabhat, J. Bacmeister, C.-T. Chen, C. Paciorek, P. J. Gleckler, K. R. Sperber, W. D. Collins, A. Gettelman, and C. Jablonowski, 2014: The effect of horizontal resolution on simulation quality in the Community Atmospheric Model, CAM5.1. Journal of Advances in Modeling Earth Systems, 6, 980–997, doi:10.1002/2013MS000276. ↩
- Westra, S., L. V. Alexander, and F. W. Zwiers, 2013: Global increasing trends in annual maximum daily precipitation. Journal of Climate, 26, 3904–3918, doi:10.1175/JCLI-D-12-00502.1. ↩
- Wright, D. M., D. J. Posselt, and A. L. Steiner, 2013: Sensitivity of lake-effect snowfall to lake ice cover and temperature in the Great Lakes region. Monthly Weather Review, 141, 670–689, doi:10.1175/mwr-d-12-00038.1. ↩
- Zhang, X., H. Wan, F. W. Zwiers, G. C. Hegerl, and S.-K. Min, 2013: Attributing intensification of precipitation extremes to human influence. Geophysical Research Letters, 40, 5252–5257, doi:10.1002/grl.51010. ↩
- van der Wiel, K., S. B. Kapnick, G. J. van Oldenborgh, K. Whan, S. Philip, G. A. Vecchi, R. K. Singh, J. Arrighi, and H. Cullen, 2017: Rapid attribution of the August 2016 flood-inducing extreme precipitation in south Louisiana to climate change. Hydrology and Earth System Sciences, 21, 897–921, doi:10.5194/hess-21-897-2017. ↩